Toward optical coherence tomography on a chip: in vivo three-dimensional human retinal imaging using photonic integrated circuit-based arrayed waveguide gratings
- PMID: 33402664
- PMCID: PMC7785745
- DOI: 10.1038/s41377-020-00450-0
Toward optical coherence tomography on a chip: in vivo three-dimensional human retinal imaging using photonic integrated circuit-based arrayed waveguide gratings
Abstract
In this work, we present a significant step toward in vivo ophthalmic optical coherence tomography and angiography on a photonic integrated chip. The diffraction gratings used in spectral-domain optical coherence tomography can be replaced by photonic integrated circuits comprising an arrayed waveguide grating. Two arrayed waveguide grating designs with 256 channels were tested, which enabled the first chip-based optical coherence tomography and angiography in vivo three-dimensional human retinal measurements. Design 1 supports a bandwidth of 22 nm, with which a sensitivity of up to 91 dB (830 µW) and an axial resolution of 10.7 µm was measured. Design 2 supports a bandwidth of 48 nm, with which a sensitivity of 90 dB (480 µW) and an axial resolution of 6.5 µm was measured. The silicon nitride-based integrated optical waveguides were fabricated with a fully CMOS-compatible process, which allows their monolithic co-integration on top of an optoelectronic silicon chip. As a benchmark for chip-based optical coherence tomography, tomograms generated by a commercially available clinical spectral-domain optical coherence tomography system were compared to those acquired with on-chip gratings. The similarities in the tomograms demonstrate the significant clinical potential for further integration of optical coherence tomography on a chip system.
Conflict of interest statement
M.S. and J.K. are employed by AMS AG but were remunerated by the framework of project COHESION, No. 848588, funded by the Austrian Research Promotion Agency (FFG). To the best of our knowledge, the named authors have no conflicts of interest, financial or otherwise.
Figures
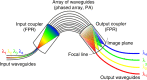
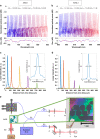
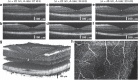
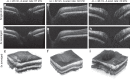
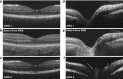
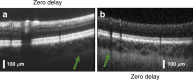
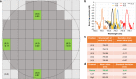
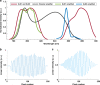
Similar articles
-
Photonic integrated Mach-Zehnder interferometer with an on-chip reference arm for optical coherence tomography.Biomed Opt Express. 2014 Mar 3;5(4):1050-61. doi: 10.1364/BOE.5.001050. eCollection 2014 Apr 1. Biomed Opt Express. 2014. PMID: 24761288 Free PMC article.
-
In vivo human retinal swept source optical coherence tomography and angiography at 830 nm with a CMOS compatible photonic integrated circuit.Sci Rep. 2021 Oct 26;11(1):21052. doi: 10.1038/s41598-021-00637-4. Sci Rep. 2021. PMID: 34702941 Free PMC article.
-
Three-dimensional (3D) monolithically integrated photodetector and WDM receiver based on bulk silicon wafer.Opt Express. 2014 Aug 11;22(16):19546-54. doi: 10.1364/OE.22.019546. Opt Express. 2014. PMID: 25321037
-
III-V-on-Silicon Photonic Integrated Circuits for Spectroscopic Sensing in the 2-4 μm Wavelength Range.Sensors (Basel). 2017 Aug 4;17(8):1788. doi: 10.3390/s17081788. Sensors (Basel). 2017. PMID: 28777291 Free PMC article. Review.
-
Additive 3D photonic integration that is CMOS compatible.Nanotechnology. 2023 May 24;34(32). doi: 10.1088/1361-6528/acd0b5. Nanotechnology. 2023. PMID: 37105145 Review.
Cited by
-
A Review: Laser Interference Lithography for Diffraction Gratings and Their Applications in Encoders and Spectrometers.Sensors (Basel). 2024 Oct 14;24(20):6617. doi: 10.3390/s24206617. Sensors (Basel). 2024. PMID: 39460098 Free PMC article. Review.
-
Enhanced medical diagnosis for dOCTors: a perspective of optical coherence tomography.J Biomed Opt. 2021 Oct;26(10):100601. doi: 10.1117/1.JBO.26.10.100601. J Biomed Opt. 2021. PMID: 34672145 Free PMC article. Review.
-
OCT on a chip aims at high-quality retinal imaging.Light Sci Appl. 2021 Jan 21;10(1):21. doi: 10.1038/s41377-021-00467-z. Light Sci Appl. 2021. PMID: 33479194 Free PMC article.
-
Precise photoelectrochemical tuning of semiconductor microdisk lasers.Adv Photonics. 2023 Sep;5(5):056004. doi: 10.1117/1.ap.5.5.056004. Epub 2023 Sep 1. Adv Photonics. 2023. PMID: 38993283 Free PMC article.
-
Exploration for adequate non-diffractive beam generation in dense scattering media.Sci Rep. 2022 May 25;12(1):8824. doi: 10.1038/s41598-022-12810-4. Sci Rep. 2022. PMID: 35614163 Free PMC article.
References
-
- Drexler, W. & Fujimoto, J. G. Optical Coherence Tomography-Technology and Applications. (Cham: Springer International Publishing, 2015).
-
- Chopra R, Keane P. Optical coherence tomography-reinventing the eye examination. Eye News. 2016;23:3.
Grants and funding
- 848588/Österreichische Forschungsförderungsgesellschaft (Austrian Research Promotion Agency)
- 848588/Österreichische Forschungsförderungsgesellschaft (Austrian Research Promotion Agency)
- 848588/Österreichische Forschungsförderungsgesellschaft (Austrian Research Promotion Agency)
- 848588/Österreichische Forschungsförderungsgesellschaft (Austrian Research Promotion Agency)
- 848588/Österreichische Forschungsförderungsgesellschaft (Austrian Research Promotion Agency)
- 848588/Österreichische Forschungsförderungsgesellschaft (Austrian Research Promotion Agency)
- 848588/Österreichische Forschungsförderungsgesellschaft (Austrian Research Promotion Agency)
- 848588/Österreichische Forschungsförderungsgesellschaft (Austrian Research Promotion Agency)
- 732969/EC | Horizon 2020 Framework Programme (EU Framework Programme for Research and Innovation H2020)
- 732969/EC | Horizon 2020 Framework Programme (EU Framework Programme for Research and Innovation H2020)
LinkOut - more resources
Full Text Sources
Other Literature Sources
Research Materials
Miscellaneous