Structure of the lipoprotein lipase-GPIHBP1 complex that mediates plasma triglyceride hydrolysis
- PMID: 30559189
- PMCID: PMC6358717
- DOI: 10.1073/pnas.1817984116
Structure of the lipoprotein lipase-GPIHBP1 complex that mediates plasma triglyceride hydrolysis
Abstract
Lipoprotein lipase (LPL) is responsible for the intravascular processing of triglyceride-rich lipoproteins. The LPL within capillaries is bound to GPIHBP1, an endothelial cell protein with a three-fingered LU domain and an N-terminal intrinsically disordered acidic domain. Loss-of-function mutations in LPL or GPIHBP1 cause severe hypertriglyceridemia (chylomicronemia), but structures for LPL and GPIHBP1 have remained elusive. Inspired by our recent discovery that GPIHBP1's acidic domain preserves LPL structure and activity, we crystallized an LPL-GPIHBP1 complex and solved its structure. GPIHBP1's LU domain binds to LPL's C-terminal domain, largely by hydrophobic interactions. Analysis of electrostatic surfaces revealed that LPL contains a large basic patch spanning its N- and C-terminal domains. GPIHBP1's acidic domain was not defined in the electron density map but was positioned to interact with LPL's large basic patch, providing a likely explanation for how GPIHBP1 stabilizes LPL. The LPL-GPIHBP1 structure provides insights into mutations causing chylomicronemia.
Keywords: GPIHBP1; X-ray crystallography; lipase; lipoproteins; triglycerides.
Copyright © 2019 the Author(s). Published by PNAS.
Conflict of interest statement
Conflict of interest statement: B.D., B.S.-L., C.Q.P., and M.M. are employees of Shire and hold stock and stock options in Shire. R.Z. and S.G.Y. are coordinators on a Leducq Transatlantic Network Grant. They have not collaborated directly on this project.
Figures
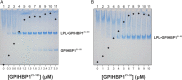
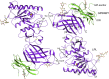
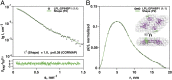
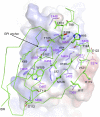
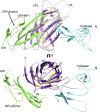
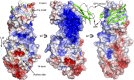
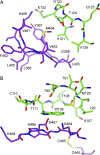
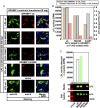
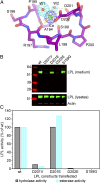
Comment in
-
Intravascular triglyceride lipolysis becomes crystal clear.Proc Natl Acad Sci U S A. 2019 Jan 29;116(5):1480-1482. doi: 10.1073/pnas.1820330116. Epub 2019 Jan 4. Proc Natl Acad Sci U S A. 2019. PMID: 30610175 Free PMC article. No abstract available.
Similar articles
-
A protein of capillary endothelial cells, GPIHBP1, is crucial for plasma triglyceride metabolism.Proc Natl Acad Sci U S A. 2022 Sep 6;119(36):e2211136119. doi: 10.1073/pnas.2211136119. Epub 2022 Aug 29. Proc Natl Acad Sci U S A. 2022. PMID: 36037340 Free PMC article.
-
A disordered acidic domain in GPIHBP1 harboring a sulfated tyrosine regulates lipoprotein lipase.Proc Natl Acad Sci U S A. 2018 Jun 26;115(26):E6020-E6029. doi: 10.1073/pnas.1806774115. Epub 2018 Jun 13. Proc Natl Acad Sci U S A. 2018. PMID: 29899144 Free PMC article.
-
Electrostatic sheathing of lipoprotein lipase is essential for its movement across capillary endothelial cells.J Clin Invest. 2022 Mar 1;132(5):e157500. doi: 10.1172/JCI157500. J Clin Invest. 2022. PMID: 35229724 Free PMC article.
-
GPIHBP1 and Lipoprotein Lipase, Partners in Plasma Triglyceride Metabolism.Cell Metab. 2019 Jul 2;30(1):51-65. doi: 10.1016/j.cmet.2019.05.023. Cell Metab. 2019. PMID: 31269429 Free PMC article. Review.
-
GPIHBP1 and Plasma Triglyceride Metabolism.Trends Endocrinol Metab. 2016 Jul;27(7):455-469. doi: 10.1016/j.tem.2016.04.013. Epub 2016 May 14. Trends Endocrinol Metab. 2016. PMID: 27185325 Free PMC article. Review.
Cited by
-
Macromolecular Interactions of Lipoprotein Lipase (LPL).Subcell Biochem. 2024;104:139-179. doi: 10.1007/978-3-031-58843-3_8. Subcell Biochem. 2024. PMID: 38963487 Review.
-
THP-1 macrophage cholesterol efflux is impaired by palmitoleate through Akt activation.PLoS One. 2020 May 21;15(5):e0233180. doi: 10.1371/journal.pone.0233180. eCollection 2020. PLoS One. 2020. PMID: 32437392 Free PMC article.
-
Targeting host-specific metabolic pathways-opportunities and challenges for anti-infective therapy.Front Mol Biosci. 2024 Feb 22;11:1338567. doi: 10.3389/fmolb.2024.1338567. eCollection 2024. Front Mol Biosci. 2024. PMID: 38455763 Free PMC article. Review.
-
Advances in fatty acids nutrition in dairy cows: from gut to cells and effects on performance.J Anim Sci Biotechnol. 2020 Nov 16;11(1):110. doi: 10.1186/s40104-020-00512-8. J Anim Sci Biotechnol. 2020. PMID: 33292523 Free PMC article. Review.
-
The structural basis for monoclonal antibody 5D2 binding to the tryptophan-rich loop of lipoprotein lipase.J Lipid Res. 2020 Oct;61(10):1347-1359. doi: 10.1194/jlr.RA120000993. Epub 2020 Jul 20. J Lipid Res. 2020. PMID: 32690595 Free PMC article.
References
-
- Korn ED. Clearing factor, a heparin-activated lipoprotein lipase. II. Substrate specificity and activation of coconut oil. J Biol Chem. 1955;215:15–26. - PubMed
-
- Korn ED. Clearing factor, a heparin-activated lipoprotein lipase. I. Isolation and characterization of the enzyme from normal rat heart. J Biol Chem. 1955;215:1–14. - PubMed
-
- Havel RJ. Triglyceride-rich lipoproteins and plasma lipid transport. Arterioscler Thromb Vasc Biol. 2010;30:9–19. - PubMed
Publication types
MeSH terms
Substances
Associated data
- Actions
Grants and funding
LinkOut - more resources
Full Text Sources
Molecular Biology Databases
Miscellaneous