The Development, Commercialization, and Impact of Optical Coherence Tomography
- PMID: 27409459
- PMCID: PMC4968928
- DOI: 10.1167/iovs.16-19963
The Development, Commercialization, and Impact of Optical Coherence Tomography
Abstract
This review was written for the special issue of IOVS to describe the history of optical coherence tomography (OCT) and its evolution from a nonscientific, historic perspective. Optical coherence tomography has become a standard of care in ophthalmology, providing real-time information on structure and function - diagnosing disease, evaluating progression, and assessing response to therapy, as well as helping to understand disease pathogenesis and create new therapies. Optical coherence tomography also has applications in multiple clinical specialties, fundamental research, and manufacturing. We review the early history of OCT describing how research and development evolves and the important role of multidisciplinary collaboration and expertise. Optical coherence tomography had its origin in femtosecond optics, but used optical communications technologies and required advanced engineering for early OCT prototypes, clinical feasibility studies, entrepreneurship, and corporate development in order to achieve clinical acceptance and clinical impact. Critical advances were made by early career researchers, clinician scientists, engineering experts, and business leaders, which enabled OCT to have a worldwide impact on health care. We introduce the concept of an "ecosystem" consisting of research, government funding, collaboration and competition, clinical studies, innovation, entrepreneurship and industry, and impact - all of which must work synergistically. The process that we recount is long and challenging, but it is our hope that it might inspire early career professionals in science, engineering, and medicine, and that the clinical and research community will find this review of interest.
Figures
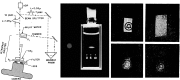
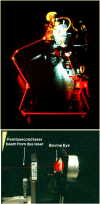
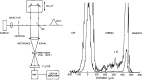
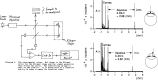
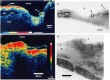
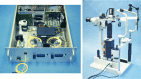
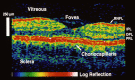
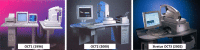
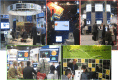
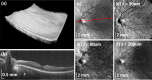
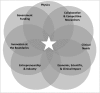
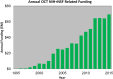
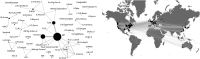
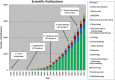
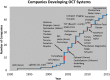
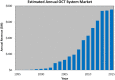
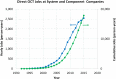
Similar articles
-
Optical coherence tomography: a review of clinical development from bench to bedside.J Biomed Opt. 2007 Sep-Oct;12(5):051403. doi: 10.1117/1.2793736. J Biomed Opt. 2007. PMID: 17994864 Review.
-
The ecosystem that powered the translation of OCT from fundamental research to clinical and commercial impact [Invited].Biomed Opt Express. 2017 Feb 21;8(3):1638-1664. doi: 10.1364/BOE.8.001638. eCollection 2017 Mar 1. Biomed Opt Express. 2017. PMID: 28663854 Free PMC article.
-
Enhanced medical diagnosis for dOCTors: a perspective of optical coherence tomography.J Biomed Opt. 2021 Oct;26(10):100601. doi: 10.1117/1.JBO.26.10.100601. J Biomed Opt. 2021. PMID: 34672145 Free PMC article. Review.
-
Applications of optical coherence tomography to cardiac and musculoskeletal diseases: bench to bedside?J Biomed Opt. 2007 Sep-Oct;12(5):051705. doi: 10.1117/1.2795689. J Biomed Opt. 2007. PMID: 17994878 Review.
-
Combining optical coherence tomography with visual field data to rapidly detect disease progression in glaucoma: a diagnostic accuracy study.Health Technol Assess. 2018 Jan;22(4):1-106. doi: 10.3310/hta22040. Health Technol Assess. 2018. PMID: 29384083 Free PMC article. Clinical Trial.
Cited by
-
Combined Rotating Ultra-High-Resolution Spectral Domain OCT and Scheimpflug Imaging for In Vivo Corneal Optical Biopsy.Diagnostics (Basel). 2024 Jul 8;14(13):1455. doi: 10.3390/diagnostics14131455. Diagnostics (Basel). 2024. PMID: 39001345 Free PMC article.
-
Retinal nerve fiber layer changes in migraine: A protocol for systematic review and meta-analysis.Medicine (Baltimore). 2020 Aug 14;99(33):e21680. doi: 10.1097/MD.0000000000021680. Medicine (Baltimore). 2020. PMID: 32872037 Free PMC article.
-
Non-steroidal anti-inflammatory agents for treating cystoid macular edema following cataract surgery.Cochrane Database Syst Rev. 2022 Dec 15;12(12):CD004239. doi: 10.1002/14651858.CD004239.pub4. Cochrane Database Syst Rev. 2022. PMID: 36520144 Free PMC article. Review.
-
Methods for objective assessment of skin involvement in systemic sclerosis.Curr Opin Rheumatol. 2023 Nov 1;35(6):301-308. doi: 10.1097/BOR.0000000000000968. Epub 2023 Aug 21. Curr Opin Rheumatol. 2023. PMID: 37605869 Free PMC article. Review.
-
Toward a New Staging System for Diabetic Retinopathy Using Wide Field Swept-Source Optical Coherence Tomography Angiography.Curr Diab Rep. 2021 Aug 26;21(9):28. doi: 10.1007/s11892-021-01401-8. Curr Diab Rep. 2021. PMID: 34448072 Review.
References
-
- Duguay MA. Light photographed in flight. American Scientist. 1971; 59: 551–556.
-
- Duguay MA,, Mattick AT. Ultrahigh speed photography of picosecond light pulses and echoes. Appl Opt. 1971; 10: 2162–2170. - PubMed
-
- Fork RL,, Greene BI,, Shank CV. Generation of optical pulses shorter than 0.1 psec by colliding pulse mode locking. Appl Phys Lett. 1981; 38: 671–673.
-
- Birngruber R,, Puliafito CA,, Gawande A,, Lin WZ,, Schoenlein RW,, Fujimoto JG. Femtosecond laser-tissue interactions: retinal injury studies. IEEE J Quantum Electron. 1987; 23: 1836–1844.
-
- Stern D,, Lin WZ,, Puliafito CA,, Fujimoto JG. Femtosecond optical ranging of corneal incision depth. Invest Ophthalmol Vis Sci. 1989; 30: 99–104. - PubMed
Publication types
MeSH terms
Grants and funding
LinkOut - more resources
Full Text Sources
Other Literature Sources