Nutrient sensing by the mitochondrial transcription machinery dictates oxidative phosphorylation
- PMID: 24430182
- PMCID: PMC4381729
- DOI: 10.1172/JCI69413
Nutrient sensing by the mitochondrial transcription machinery dictates oxidative phosphorylation
Abstract
Sirtuin 3 (SIRT3), an important regulator of energy metabolism and lipid oxidation, is induced in fasted liver mitochondria and implicated in metabolic syndrome. In fasted liver, SIRT3-mediated increases in substrate flux depend on oxidative phosphorylation (OXPHOS), but precisely how OXPHOS meets the challenge of increased substrate oxidation in fasted liver remains unclear. Here, we show that liver mitochondria in fasting mice adapt to the demand of increased substrate oxidation by increasing their OXPHOS efficiency. In response to cAMP signaling, SIRT3 deacetylated and activated leucine-rich protein 130 (LRP130; official symbol, LRPPRC), promoting a mitochondrial transcriptional program that enhanced hepatic OXPHOS. Using mass spectrometry, we identified SIRT3-regulated lysine residues in LRP130 that generated a lysine-to-arginine (KR) mutant of LRP130 that mimics deacetylated protein. Compared with wild-type LRP130 protein, expression of the KR mutant increased mitochondrial transcription and OXPHOS in vitro. Indeed, even when SIRT3 activity was abolished, activation of mitochondrial transcription and OXPHOS by the KR mutant remained robust, further highlighting the contribution of LRP130 deacetylation to increased OXPHOS in fasted liver. These data establish a link between nutrient sensing and mitochondrial transcription that regulates OXPHOS in fasted liver and may explain how fasted liver adapts to increased substrate oxidation.
Figures
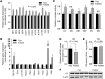
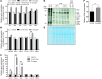
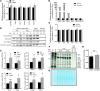
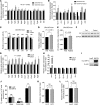
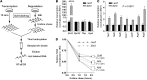
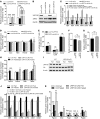
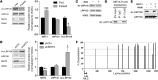
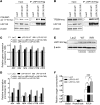
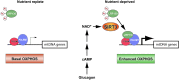
Similar articles
-
Fiber-specific and whole-muscle LRP130 expression in rested, exercised, and fasted human skeletal muscle.Pflugers Arch. 2020 Mar;472(3):375-384. doi: 10.1007/s00424-020-02359-4. Epub 2020 Feb 17. Pflugers Arch. 2020. PMID: 32065259
-
LRP130 protein remodels mitochondria and stimulates fatty acid oxidation.J Biol Chem. 2011 Dec 2;286(48):41253-41264. doi: 10.1074/jbc.M111.276121. Epub 2011 Oct 4. J Biol Chem. 2011. PMID: 21971050 Free PMC article.
-
SIRT3 regulates mitochondrial fatty-acid oxidation by reversible enzyme deacetylation.Nature. 2010 Mar 4;464(7285):121-5. doi: 10.1038/nature08778. Nature. 2010. PMID: 20203611 Free PMC article.
-
Dual control of mitochondrial biogenesis by sirtuin 1 and sirtuin 3.Mitochondrion. 2013 Nov;13(6):755-61. doi: 10.1016/j.mito.2013.04.002. Epub 2013 Apr 11. Mitochondrion. 2013. PMID: 23583953 Review.
-
Protein Lysine Acetylation: Grease or Sand in the Gears of β-Cell Mitochondria?J Mol Biol. 2020 Mar 6;432(5):1446-1460. doi: 10.1016/j.jmb.2019.09.011. Epub 2019 Oct 16. J Mol Biol. 2020. PMID: 31628953 Review.
Cited by
-
Mechanisms of mitochondrial respiratory adaptation.Nat Rev Mol Cell Biol. 2022 Dec;23(12):817-835. doi: 10.1038/s41580-022-00506-6. Epub 2022 Jul 8. Nat Rev Mol Cell Biol. 2022. PMID: 35804199 Free PMC article. Review.
-
Fiber-specific and whole-muscle LRP130 expression in rested, exercised, and fasted human skeletal muscle.Pflugers Arch. 2020 Mar;472(3):375-384. doi: 10.1007/s00424-020-02359-4. Epub 2020 Feb 17. Pflugers Arch. 2020. PMID: 32065259
-
The RNA-binding protein LRPPRC promotes resistance to CDK4/6 inhibition in lung cancer.Nat Commun. 2023 Jul 14;14(1):4212. doi: 10.1038/s41467-023-39854-y. Nat Commun. 2023. PMID: 37452037 Free PMC article.
-
Role of SIRT3 in neurological diseases and rehabilitation training.Metab Brain Dis. 2023 Jan;38(1):69-89. doi: 10.1007/s11011-022-01111-4. Epub 2022 Nov 14. Metab Brain Dis. 2023. PMID: 36374406 Free PMC article. Review.
-
The effect of acute and chronic sprint-interval training on LRP130, SIRT3, and PGC-1α expression in human skeletal muscle.Physiol Rep. 2016 Sep;4(17):e12879. doi: 10.14814/phy2.12879. Physiol Rep. 2016. PMID: 27604398 Free PMC article.
References
Publication types
MeSH terms
Substances
Grants and funding
LinkOut - more resources
Full Text Sources
Other Literature Sources
Molecular Biology Databases