Ribonuclease III mechanisms of double-stranded RNA cleavage
- PMID: 24124076
- PMCID: PMC3867540
- DOI: 10.1002/wrna.1195
Ribonuclease III mechanisms of double-stranded RNA cleavage
Abstract
Double-stranded(ds) RNA has diverse roles in gene expression and regulation, host defense, and genome surveillance in bacterial and eukaryotic cells. A central aspect of dsRNA function is its selective recognition and cleavage by members of the ribonuclease III (RNase III) family of divalent-metal-ion-dependent phosphodiesterases. The processing of dsRNA by RNase III family members is an essential step in the maturation and decay of coding and noncoding RNAs, including miRNAs and siRNAs. RNase III, as first purified from Escherichia coli, has served as a biochemically well-characterized prototype, and other bacterial orthologs provided the first structural information. RNase III family members share a unique fold (RNase III domain) that can dimerize to form a structure that binds dsRNA and cleaves phosphodiesters on each strand, providing the characteristic 2 nt, 3'-overhang product ends. Ongoing studies are uncovering the functions of additional domains, including, inter alia, the dsRNA-binding and PAZ domains that cooperate with the RNase III domain to select target sites, regulate activity, confer processivity, and support the recognition of structurally diverse substrates. RNase III enzymes function in multicomponent assemblies that are regulated by diverse inputs, and at least one RNase III-related polypeptide can function as a noncatalytic, dsRNA-binding protein. This review summarizes the current knowledge of the mechanisms of catalysis and target site selection of RNase III family members, and also addresses less well understood aspects of these enzymes and their interactions with dsRNA.
© 2013 The Authors. WIREs RNA published by John Wiley & Sons, Ltd.
Figures
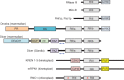
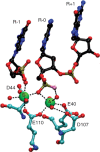
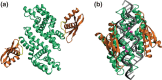
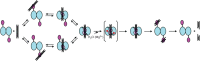
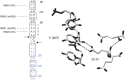
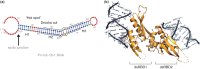
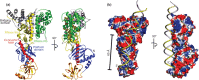
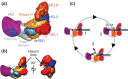
Similar articles
-
New approaches to understanding double-stranded RNA processing by ribonuclease III purification and assays of homodimeric and heterodimeric forms of RNase III from bacterial extremophiles and mesophiles.Methods Enzymol. 2008;447:119-29. doi: 10.1016/S0076-6879(08)02207-6. Methods Enzymol. 2008. PMID: 19161841
-
Intrinsic double-stranded-RNA processing activity of Escherichia coli ribonuclease III lacking the dsRNA-binding domain.Biochemistry. 2001 Dec 11;40(49):14976-84. doi: 10.1021/bi011570u. Biochemistry. 2001. PMID: 11732918
-
Noncatalytic assembly of ribonuclease III with double-stranded RNA.Structure. 2004 Mar;12(3):457-66. doi: 10.1016/j.str.2004.02.004. Structure. 2004. PMID: 15016361
-
Structural basis for non-catalytic and catalytic activities of ribonuclease III.Acta Crystallogr D Biol Crystallogr. 2006 Aug;62(Pt 8):933-40. doi: 10.1107/S090744490601153X. Epub 2006 Jul 18. Acta Crystallogr D Biol Crystallogr. 2006. PMID: 16855311 Review.
-
The mechanism of RNase III action: how dicer dices.Curr Top Microbiol Immunol. 2008;320:99-116. doi: 10.1007/978-3-540-75157-1_5. Curr Top Microbiol Immunol. 2008. PMID: 18268841 Review.
Cited by
-
Mutually exclusive RNA secondary structures regulate translation initiation of DinQ in Escherichia coli.RNA. 2016 Nov;22(11):1739-1749. doi: 10.1261/rna.058461.116. Epub 2016 Sep 20. RNA. 2016. PMID: 27651528 Free PMC article.
-
Dynamical comparison between Drosha and Dicer reveals functional motion similarities and dissimilarities.PLoS One. 2019 Dec 10;14(12):e0226147. doi: 10.1371/journal.pone.0226147. eCollection 2019. PLoS One. 2019. PMID: 31821368 Free PMC article.
-
Designing of RNA Molecule Translating for Activitable Melittin as Selective Targeting of Leishmania Infected Cells.Iran J Parasitol. 2021 Jul-Sep;16(3):443-453. doi: 10.18502/ijpa.v16i3.7098. Iran J Parasitol. 2021. PMID: 34630590 Free PMC article.
-
Determination of relative rate constants for in vitro RNA processing reactions by internal competition.Anal Biochem. 2014 Dec 15;467:54-61. doi: 10.1016/j.ab.2014.08.022. Epub 2014 Aug 28. Anal Biochem. 2014. PMID: 25173512 Free PMC article.
-
Mapping of internal monophosphate 5' ends of Bacillus subtilis messenger RNAs and ribosomal RNAs in wild-type and ribonuclease-mutant strains.Nucleic Acids Res. 2016 Apr 20;44(7):3373-89. doi: 10.1093/nar/gkw073. Epub 2016 Feb 15. Nucleic Acids Res. 2016. PMID: 26883633 Free PMC article.
References
-
- Haines DS, Strauss KI, Gillespie DH. Cellular response to double-stranded RNA. J Cell Biochem. 1991;46:9–20. - PubMed
-
- Nicholson AW. Structure, reactivity and biology of double-stranded RNA. Prog Nucleic Acid Res Mol Biol. 1996;52:1–65. - PubMed
-
- deFaria IJ, Olmo RP, Silva EG, Marques JT. dsRNA sensing during viral infection: lessons from plants, worms, insects, and mammals. J. Interferon Cytokine Res. 2013;33:239–253. - PubMed
Publication types
MeSH terms
Substances
Grants and funding
LinkOut - more resources
Full Text Sources
Other Literature Sources
Molecular Biology Databases
Research Materials